Developing a framework for Multiphysics modeling of High-Temperature Electrolysis
In the context of climate change mitigation, hydrogen represents a promising and versatile energy vector. Water electrolysis powered by renewable energy offers a sustainable method for hydrogen production, with efficiency gains achievable at the higher operating temperatures of Solid Oxide Electrolysis Cells (SOECs). Further integration into P2X scenarios can be realized via high-temperature co-electrolysis of H2O and CO2, enabling de-fossilization of syngas production. A theoretical, steady-state model was developed to elucidate the fundamentals of the electrolysis process, considering the simultaneous occurrence of thermodynamics, kinetics, heat transfer, chemistry, and diffusion processes within the cell using COMSOL Multiphysics®. This model also examined the influence of material parameters including porosity, tortuosity, conductivity, and thermal transport properties across each cell layer. The simplified, real-scale SOEC model (Figures 1 and 2) describes an experimentally utilized button cell and provides physical and chemical descriptions of each layer. The cell model includes an (La,Sr)(Co(,Fe))O3 (LSC(F)) air electrode, an 8 mol% Y2O3 stabilized ZrO2 (8YSZ) electrolyte, and a Ni-8YSZ cermet fuel electrode divided into functional and bulk layers, mimicking commercially available state-of-the-art cells. The only simplification compared to the real cell geometry is the omission of the Gd2O3 doped CeO2 (GDC) barrier layer, which is accounted for by an enlarged electrolyte layer. Using COMSOL’s Water Electrolyzer and Chemistry modules, the model incorporates electrochemical reactions at the triple-phase boundary with Butler-Volmer kinetics, and transport in porous media and gas channels as described by the Brinkman Equation. Species interactions are approximated by binary Maxwell-Stefan diffusion constants to account for effects arising from the heat of reaction and material properties. In the case of CO2 or co-electrolysis, the Boudouard equilibrium and the reverse water-gas shift reaction were also considered. This framework facilitated the model’s validation using data for extreme cases of CO2 and steam electrolysis, supported by corresponding experimental data. The validation of electrochemical parameters was achieved using i-V-characteristics (Figure 3), while impedance spectra calculations provided additional insights into the model's mechanisms (Figure 4). This approach allows a deeper understanding of the ongoing processes and dominant limitations in the respective extreme cases. From the analysis of i-V-characteristics and sensitivity analyses, it was demonstrated that the exchange current density (i0) for steam electrolysis aligns with experimentally determined values. Conversely, for CO2 electrolysis, i0 is found to be one order of magnitude lower to match experimental i-V-characteristics at higher current densities. Based on these extreme cases, the combined case of co-electrolysis can be modeled too. Unlike the exchange current density, gas flow rate and gas permeability were identified as non-sensitive parameters. The developed model framework allows for further investigations, particularly in terms of parameter variations. In light of SOEC’s current stability issues, this approach allows elucidating the origin and mechanisms of cell degradation being the primary obstacle to SOEC commercialization. Degradation studies can be implemented with time-dependent solutions to account for the gradual voltage increase over time. Considering microstructural parameters further allows to investigate the impact of microstructural changes, which are suspected to be significant contributors to overall degradation. A related study is currently underway and has highlighted further refinement possibilities for the model framework.
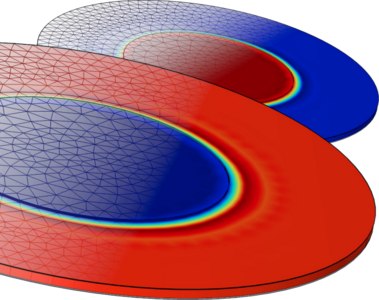
Download
- eyckeler_10201_poster.pdf - 3.06MB